Here's an interesting mental math challenge. Given two different positive real numbers, which we'll call a and b, which is greater, ab or ba? If you're able to calculate both exponents mentally, such as 23 vs 32, then that's probably the simplest way to go. What happens if one or both exponential expressions are too hard to mentally calculate?
To solve this, we'll need to find a general rule. Some advanced calculation will be required to find it. However, once we do the work to find the rule, you'll see that almost no math will be needed to solve these types of problems!
SEARCH FOR THE RULE: Consider that 23 < 32, but 34 > 43. Just looking at the placement of smaller and bigger numbers, there doesn't seem to hard and fast rule which applies. The best place to start is just by assuming what we wish to discover:
From that point, let's see if we can separate a and b somehow. The quickest way is to raise both sides to the power of 1/ab:
In English, then, when the ath root of a is greater than the bth root of b, then ab > ba. Don't worry, though. You won't have to do any roots in your head. Instead, just look at this Desmos graph where y=xth root of x. If you click in the box marked 1 at the upper left, Desmos highlights two points, the point with the minimum y value, which is (0, 0), and the point with the maximum value, (2.718, 1.445).
Wait a minute! The value of 2.718 sounds familiar. Could the maximum value of xth root of x really be e? Sure enough, Wolfram|Alpha verifies that the maximum is e!
Look at both sides of the graph, then. From 0 to e, the graph increases. From e on upwards, the value of the xth root of x will steadily decrease. In fact, the value will just keep getting closer and closer to 1.
This means we've found the start of our rule. When you have two numbers, both of which are equal to or greater than e, the smaller number x will always yield a greater xth root of x than the higher number. This tells us that the ath root of a is greater than the bth root of b when a is the smaller number. Working backwards to our original assumption, this means that:
When a and b are both equal to or greater than e, ab is always greater than ba when a is the smaller number and b is the larger number.
There's our rule!
WORKING WITH INTEGERS: What about when one or both of a and b are less than e? In the case of positive integers, this means we only have to consider the cases involving 1 and 2.
The case of 1 is easy. Assume that a equals 1 and b has a value of 2 or more. 1b will always be 1, and b1 will always be b, which is 2 or more. That covers every case involving 1.
What about 2? Let's work through each case individually, always assigning a to a value of 2, and b a value other than 2. If b is 1, we just covered that case. b can't be 2, as it would be the same as a. What about when b is 3? We already covered the fact that 23 < 32, so we remember that special case. What happens when b is 4? This is a very unique case, as 24 is exactly equal to 42! In fact, this is a well-known special case, as it's the only time when ab equals ba when a and b are different integers. When b is 5 ore greater, as you can see in this graph, we can fall back on the rule we set above.
A CLASSIC CHALLENGE: Which is greater, πe or eπ? There's a problem you probably never thought you'd solve in your head!
Let's go see if our primary rule applies. Are a and b both equal to or greater than e? e, of course, is exactly equal to e (2.71828 and so on). π, as any Grey Matters readers should already know, is roughly 3.1415, and therefore also greater than e.
This means we can apply our primary rule! e is smaller than π, which means that eπ must be greater than πe. A quick verification with Wolfram|Alpha shows that this is correct.
About 4 years ago, Presh Talwalkar wrote up several approaches to this classic problem. Interestingly, this approach wasn't included. The 4th method does work with the xth root of x as we did, but it uses a deeper approach involving calculus.
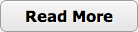